FOR all its marvels, electricity frequently disappoints. Batteries run out. Appliances overheat. Your electric utility bill inches a little higher every month.
Electricity is, alas, imperfect. Sure, electrons seem peppy enough for most of our needs, but they fail to live up to their full potential. These diminutive charge carriers drift lackadaisically through the copper wiring in our homes, bumping against impurities and wasting precious energy as heat.
The frustrating thing is that we know they can do better. Electrons are capable of superconduction: with a little coaxing they can sail effortlessly and indefinitely along a wire without losing energy. If you had superconducting circuits in your camera, you wouldn't need to replace the battery. Power cables carrying superconducting currents would hum along faithfully for longer than the lifetime of the universe. And your monthly utility bill would be less of a shock.
There is, of course, a catch. So far, such behaviour has only been spotted at temperatures so frigid that they do not occur naturally on Earth. Just a handful of modern devices exploit superconductivity, yet the need for unearthly temperatures hasn't dissuaded physicists from chasing the dream of perpetual electron motion.
That's why the discovery earlier this year of an entirely new class of superconducting materials has sent scientists into a frenzy of activity. Although these new superconductors don't break any temperature records just yet, they are breaking all the known rules of superconductivity because they are made from iron. Even better, they offer the tantalising possibility that a little tinkering could raise their operating temperatures, perhaps to as high as room temperature. They could even help us crack one of the deepest mysteries in physics by explaining how all superconductors work.
Most people had abandoned dreams of finding a unifying theory that explains how superconductors work
No one is more surprised by the frenzy than Japanese physicist Hideo Hosono, who with his team at the Tokyo Institute of Technology reported the first of these superconducting materials in February (Journal of the American Chemical Society, vol 130, p 3296). More than 110 papers have since been written on the peculiarities of these new compounds. "The present excitement was totally beyond my imagination," says Hosono.
Hosono wasn't looking for a radical new superconductivity when he made the material that caused such a stir. He was searching for a transparent semiconductor and began tinkering with a cocktail of iron, arsenic, oxygen and the rare-earth metal lanthanum. To boost the electric current, he substituted 10 per cent of the oxygen atoms with fluorine, which has an extra electron. To his amazement, he found that the material lost all its electrical resistance below 26 kelvin.
Within weeks, researchers at the Institute of Physics in Beijing had replicated Hosono's results and gone a step further. They made a version containing cerium atoms instead of lanthanum and discovered that it worked as a superconductor at 41 K. Next Xianhui Chen at the University of Science and Technology of China in Hefei replaced lanthanum with samarium and found the superconducting transition temperature climbed to 43 K. Using neodymium or praseodymium pushed the temperature up to over 50 K.
Less than two months after Hosono's publication, Zhong-Xian Zhao's group at the Institute of Physics in Beijing nudged the transition temperature up to 55 K by synthesising the samarium version under high pressure.
It's still far from the warmest superconductors ever discovered - that honour goes to the family of high-temperature superconductors that are based on copper oxide that lose all resistance at 138 K, and even higher under pressure. Even so, the rapid progress in raising the transition temperature is very promising, and these iron arsenide superconductors have plenty of other features to keep researchers busy.
What
most physicists desperately want to know is, how do the electrons in
these materials superconduct? A wealth of experiments have shown that
lanthanum oxygen iron arsenide consists of a layer of iron arsenide
sandwiched between two tiers of lanthanum oxide
From the outset, most physicists would never have suspected that a compound so rich in iron could become a superconductor. Iron is magnetic, and magnetism has long been considered the killjoy at the superconducting party, at least for superconductors that work near absolute zero.
It's all to do with the behaviour of electrons. Back in 1957, physicists John Bardeen, Leon Cooper and Robert Schrieffer showed how metals and alloys such as niobium-tin superconduct. They realised that electrons can flow without resistance if they overcome their natural repulsion for each other and hook up in so-called Cooper pairs, glued together by tiny vibrations in the metal's lattice structure. When two electrons pair up in this manner, they act as a single extended object and can move smoothly throughout the lattice.
Bring a strong magnet close to a superconductor, however, and the speedy flow of electrons resumes its usual crawl. The reason is that magnetism requires electrons to be arranged with their spins all pointing the same direction, whereas in Cooper pairs the two electrons must spin in opposite directions.
If the magnetic field is strong enough, it finds its way into the material, and can break up the pairs and destroy superconductivity (New Scientist, 7 February 1998, p 32). "If you were going to look for superconductivity anywhere, the last place you would look is in iron-based materials," says Philip Phillips, a theoretical physicist at the University of Illinois at Urbana-Champaign.
To understand what was going on, Pengcheng Dai at the University of Tennessee in Knoxville got some samples of the lanthanum compound from Zhao's lab in Beijing and enlisted the help of Jeffrey Lynn and colleagues at the National Institute of Standards and Technology's Center for Neutron Research in Gaithersburg, Maryland. As they cooled the samples down toward absolute zero, they bombarded them with neutrons to probe the structural and magnetic properties of the material.
When the temperature reached 150 K, the researchers noticed that the shape of the lattice became distorted. As the temperature continued to drop, something interesting happened around 134 K. Instead of all pointing in one direction, the electron spins on neighbouring atoms lined up in opposite directions - a phenomenon called antiferromagnetism.
Next, the team repeated the experiment using a sample doped with fluorine atoms. The extra electrons provided by the fluorine changed the profile completely. Gone were both the lattice distortion and the antiferromagnetic order; instead the doped sample lost all its resistance. Astonishingly, this pattern was similar to what happens in high-temperature copper oxide superconductors.
The results got physicists wondering whether magnetism and superconductivity are really such adversaries. Maybe magnetic order plays a necessary role in high-temperature superconductivity after all. "Almost everybody believes that spins are playing a fundamental role in the pairing, although they don't know exactly how," says Lynn.
Why does it work?
How high-temperature superconductors work is one of the biggest mysteries in physics. Discovered in 1986, these ceramics can operate at higher temperatures than had ever been seen before and sparked huge excitement. Everyone who could do so began to study them, and the transition temperature steadily climbed. "People extrapolated that by the summer of 1987 we'd up to room temperature," says Lynn.
But it never happened. Years passed and the dream of cheap electricity faded, to be replaced by a lot of hard work geared toward understanding what caused the materials to superconduct. Researchers found that it was something to do with electron pairing, yet ruled out Cooper pairs glued together by lattice vibrations. "After 20 years of the smartest people on Earth thinking about it, nobody knows yet how it works," says Robert Cava, an expert in superconductors at Princeton University.
The iron arsenide family seems to offer important new clues. While magnetic order plays a role both in copper oxides and in these new materials, they are far from identical in all respects.
One important difference lies with what physicists call "the gap", which is simply the energy required to break up the superconducting electron pairs. The larger this energy gap, the harder it is to break the pairs. At least, that's the case for conventional superconductors, as described by Bardeen, Cooper and Schrieffer's theory. Here the energy it takes to break up the pair is the same along any axis through the material. In contrast, the gap in copper oxide superconductors is downright bizarre. Instead of being the same in any direction, the gap can vary widely, so that in some directions it takes no energy to break up a pair.
What is the gap like in these new materials? Chia-Ling Chien and his colleagues at Johns Hopkins University in Baltimore, Maryland, decided to measure this quantity, which Chien calls the soul of the superconductor. "We were anticipating something quite bizarre - something no less bizarre than the copper oxide superconductor's gap," says Chien. Instead, he found that the gap was far better behaved. So much so that it resembled a low-temperature superconductor.
So these materials embody aspects of both high-temperature and conventional superconductors. "This raises the spectre that really something new is at work, and is incredibly exciting at this early stage," says Zlatko Tesanovic, a theorist at Johns Hopkins.
It's exciting because most researchers have long abandoned dreams of finding a unifying theory that would explain what happens in both conventional superconductors and the high-temperature varieties. In fact, most would be happy to settle for a theory that could describe just the copper oxides, or perhaps, on a good day, all high-temperature superconductors.
A unifying theory should exist, many physicists believe. "Superconductivity is a fairly robust feature," says Jeroen van den Brink, a condensed-matter theorist at Leiden University in the Netherlands. "This tells you that there should be a simple, basic physical mechanism driving it."
The similarities between the copper oxides and the iron-based materials suggest there could some day be a unifying theory, but not everyone is convinced. Some physicists point out that the iron arsenides have more in common with another family of superconductors called heavy fermions. Others suggest that none of these compounds work alike. "I'm reminded of a quote from Tolstoy," says Phillips. "He says that all happy families are happy in the same way, but every unhappy family is unhappy in its own way."
Whatever the mechanism, these iron-based materials are already generating excitement about their practical applications. For one thing, they can withstand strong magnetic fields better than the copper oxides. In May, researchers at Florida State University in Tallahassee reported in Nature that the magnetic field strength needed to disrupt these new superconductors is very high, of the order of 45 tesla (vol 435, p 903). That means they could be used in applications that employ superconductivity to make strong electromagnets, such as magnetic resonance imaging.
Making iron arsenide superconductors requires great skill because arsenic is both toxic and difficult to work with, and this might limit their uses. One lab in the US learned this the hard way a few months ago when a researcher caused a small explosion.
Undaunted, a group in China has already developed a preliminary set of wires from the lanthanum compound. Today's power lines lose on average 7 per cent of the current they carry, so lossless power transmission could save a fortune and reduce demand on power stations during peak hours of the day.
These applications will be far more likely to come to fruition if researchers can coax the iron arsenides to superconduct above 77 K and into the range where the materials can be cooled using liquid nitrogen, rather than pricey liquid helium.
Not surprisingly, researchers are still tinkering with the iron arsenides in an effort to improve them. Some believe that the rare-earth elements such as lanthanum play a more important role in superconductivity than previously thought. Others argue that new cocktails of rare-earth atoms will never superconduct above 55 K, and that you need to alter the crystal structure instead. Further clues might come now that scientists have grown the first single crystals of rare-earth iron arsenides, which are much purer than the polycrystalline materials synthesised so far.
Few physicists are brave enough to speculate whether iron arsenide superconductors will ever operate at room temperature. "It is like promising a cure for cancer," says van den Brink. "Researchers have learned to be very careful about that."
One thing is clear: superconductivity lurks in materials we never suspected. A thoughtful perusal of the periodic table could someday yield the miracle combination that brings it to everyday life.
Space-time to the rescue
No one knows why some materials superconduct just a few degrees above absolute zero, while others can operate at almost 140 kelvin. Could extra dimensions of space and time explain it?
Djordje Minic and Jean Heremans at Virginia Tech in Blacksburg speculate that they could. In an unpublished paper, they propose applying the theory of extra dimensions to the electrons in a superconductor (www.arxiv.org/abs/0804.2880).
Extra dimensions have been postulated by theorists to explain why gravity is so much weaker than the other fundamental forces in nature. According to these ideas, we are not aware of these extra dimensions because matter and light are trapped in a three-dimensional flatland. What makes the extra dimensions of space so peculiar is that only gravity can reach into them. It's only because it leaks out into higher dimensions that an "effective gravity" appears so feeble to us.
So what does this have to do with superconductors? High-temperature superconductors are made of layers of atoms with the electrons moving through sheets of copper oxide. Minic and Heremans liken this two-dimensional world to our three-dimensional flatland. Interactions between the layers in the superconductor could give electrons access to the equivalent of an extra dimension.
Minic and Heremans admit that they have only scratched the surface of the idea and so far haven't been able to describe in detail what happens to the electrons. However they find similarities between the equations describing electrons moving in a superconductor and those describing extra dimensions.
Catherine Zandonella is a science writer based in Princeton, New Jersey (www.catzan.org)
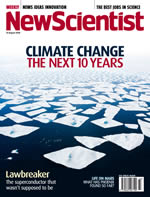
- New Scientist
- Not just a website!
- Subscribe to New Scientist and get:
- 51 issues of New Scientist magazine delivered to your door
- unlimited online access to articles from over 500 back issues
- Subscribe Now and Save
If you would like to reuse any content from New Scientist, either in print or online, please contact the syndication department first for permission. New Scientist does not own rights to photos, but there are a variety of licensing options available for use of articles and graphics we own the copyright to.
ADVERTISEMENT